Stellar-Mass Black Holes
This essay explores what we know about stellar-mass black holes and how we find them. To do this
we need to look at what a stellar-mass black hole is and how we can detect their presence. We can not view
them directly due to their nature (COSweb.)
All known stellar-mass black holes (and candidates)
are found in a binary system with normal stars. It is this association that allows us to infer the presence
of these black holes. To date 20 systems are known to contain bodies too compact to be neutron stars. There
are also another 20 systems with black hole candidates. However, vital information about these systems has
yet to be determined (Bemillard and McClintock 2006.) Since about half of stars are contained in binary
systems there is likely to be many more.
Other types of black holes include super-massive and
intermediate-mass black holes. Super-massive black holes have been found at the centre of our galaxy and
other large galaxies (COSweb.) Intermediate black holes are thought to exist and
are of a mass between stellar-mass and super-massive black holes. Primordial black holes are hypothetical objects are
thought to have resulted from the big bang. These objects are beyond the scope of this
essay.
Evolution of Stellar-Mass Black Holes Binary
Systems
Stellar-mass black holes result from the collapse of stars with masses of less than 100 solar
masses (COSweb.) The development of a black hole from such stars is the result of the exhaustion of fusion fuel
in, and around, the core of the star (Freedman and Kauffman section p. 590.) Without fusion supplying outward
pressure to counteract gravity a supernova results. If the remains are greater than the theoretical mass
limit of a neutron star (ie 2.3 solar masses) a black hole may form. Typically known stellar-mass black holes
are between 3 and 20 solar masses (COSweb.)
In close binary systems there can be a transfer of
mass from the normal star to the compact body (Bemillard and McClintock, 2006.) This may be due to tidal
forces or when the optical star swells to become a red giant near the end of its life overflowing onto the
Roche lobe of the compact companion (NOVAweb.) The material does not move directly from the star to the black
hole but swirls around it in an accretion disk. The material spirals inwards on its inevitable journey to
become part of the black hole. As the gas gets closer to the black hole’s event horizon its temperature
increases due to friction and collisions. In the final 200km the temperature increases to 10’s of million
Kelvin (COSweb.) At these temperatures the particles emit x-ray and gamma radiation (fig. 1.) It is this
radiation that we are able to detect. The presence of x-ray emissions from binary systems containing a
compact component is the best indicator of a black hole that we have. We are able to detect x-ray emissions
that originate within 90km of the event horizon of a black hole (NASAweb.)
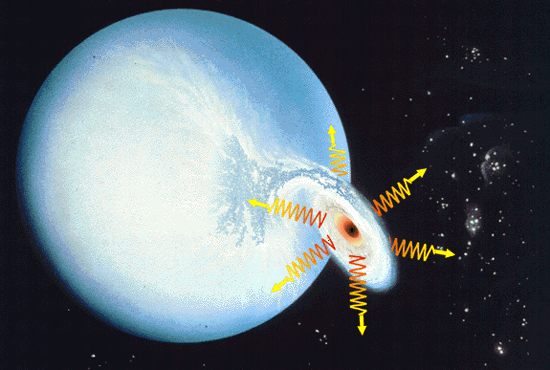
Figure 1:Illustration showing the exchange of gas from an optical binary star to its
companion black hole. The material collects in the accretion disk and spirals into the black hole due to
gravity. Friction within accretion increases temperature to a point where (in the last 200km) x-ray radiation
is emitted before the gas falls past the event horizon. (source HARVweb)
X-ray Binary System
Types
X-ray emitting binary systems containing black
holes can be divided into two classes (CAMBweb): high mass x-ray binaries (HMXB) and low mass x-ray binaries
(LMXB.) HMXBs contain optical members with masses greater than 10 solar masses (NOVAweb.) They form when the
larger of stars in the binary system reaches the end of its life resulting in a supernova and the remnants
being of more mass than the limits of a neutron star (NOVAweb.) The remaining visible star is a O or B class
star. As the smaller star nears the end of its life it enters the red giant stage with mass transfer taking
place. This is known as the HMXB stage.
Of the 20 known black hole containing binary system
three of them are consistently bright in x-ray radiation (Bemillard and McClintock 2006.) These systems
contain large O or B class optical companion stars (i.e. they are HMXB systems.) This indicates that a steady
stream of material is being accreted by the black hole (fig 2.)
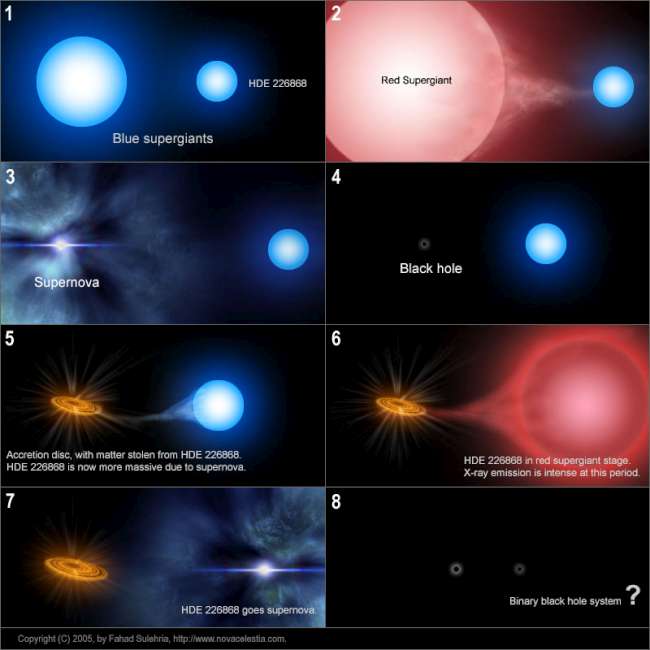
Figure 2: A model for the
development of a HMXB system (source NOVAweb)
1) Initial
system with 2 stars in binary system.
2) The larger
star approaches the end of its life and becomes a red supergiant with the smaller star
capturing gas from it.
3) The larger
companion’s life ends resulting in a supernova.
4) As the
remnant of the star is greater than 3 solar masses so a black hole
forms.
5) The black
hole captures solar wind and gas from the remaining star.
6) The star
reaches the end of its life and becomes a red giant. This results in an increased rate of
mass transfer.
7) The
remaining star forms a supernova at the end of its life.
8) If the
remnant of that star is greater than 3 solar masses it will form a black
hole.
|
LMXB systems consist of a body the same mass as our
sun or less and a black hole (or other compact body, see fig 3.) The origin of the low mass x-ray binary
systems is less clear and may be the result of a black hole capturing a star (CAMBweb.) These systems result
in a slow mass transfer and x-rays being emitted periodically in bursts.
Observations of LMXB systems have shown that the
period between individual bursts varies between the systems. The burst may last days to months and fade over
a period of a year. The optical partner has also been observed to fade after an x-ray burst. The mechanism
causing these burst is thought to be due to instabilities in the accretion disk due to insufficient flow of
material from the companion star (CAMBweb.) The x-ray emissions are softer than from LMXB systems (i.e. of
lower energy.) It is thought that the normal star overflows into the black holes Roche lobe allowing transfer
of gas (NOVAweb.)
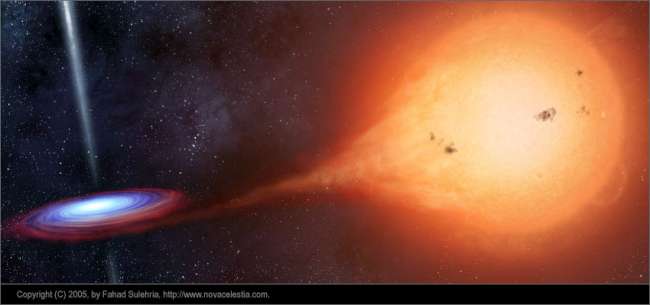
Figure 3: A LMXB system showing
mass transfer of material as the star overflows onto the black hole’s Roche lobe (source
NOVAweb.)
|
X-ray Binary System
States
There are a number of states into which x-ray binary systems are classified (McClintock and Remillard, 2004.) Three of the fives
states are quite clearly different. In the high/soft state the system is at its highest luminosity at x-ray
frequencies. X-rays originating in the accretion disk dominates the system. In the low/hard state emission
from the disk is less and dominated by emissions from the corona (termed as non-thermal emissions, Malzac,
2008.) The difference between these two states is thought to be due to the disk being cooler and withdrawn
from the black hole (Bemillard and McClintock 2006.) The
system may also be in a quiescent state in which non-thermal emissions also dominate. The remaining two
states are intermediately between the low/hard and the high/soft states
The interaction between the accretion disk and a black hole’s magnetic
field may give rise to radio jets. These jets occur when a system is in the low/hard state and material from
the accretion disk is ejected along the poles of the body’s rotation. Radio emissions observed is the result
of electrons travelling at relativistic velocities (Malzac, 2008.)
How We Detect Stellar-mass
Black Holes
There are three properties that a black hole may
possess. They are mass, electrical change and spin (Freedman and Kauffman p 594-596.) None of these
properties can be detected directly from a remote location. It is important to determine the mass of the
compact body as it is an important indicator of what the object is.
The effect that mass has on other bodies can be
determined. The gravitational effect that a black hole has on nearby bodies is a significant tool available
to astronomers. If we can determine the mass of the optical member and the orbital period of the binary it is
possible to calculate the mass of the unseen partner in the system. To achieve this we can use our knowledge
of the association between spectral type and stellar mass and use Newton’s form of Kepler’s third law to determine the mass of the compact
body.
The difficult part of determining the mass of the
black hole from Kepler’s third Law is determining the radial velocity. As these systems can not be resolved
optically we must rely on other methods. One of these methods is to investigate the spectroscopic change in
the optical object due to blue-shift (as the star move towards us) and red-shift (as it moves away.) To be
most effective the plane of the orbit needs to be directed towards the observer (LaSala, Charles, Smith et
al, 1998.) This method can determine orbital period but may also be used to determine radial velocities.
However, only the velocity towards and away from the observer can be calculated. Therefore, unless the plane
of orbit is directed towards the observer, the measured velocity will not be its true velocity. As such only
the minimum mass of the compact body can be determined if the orbit plane is
inclined.
If the accretion disk is eclipsed by the normal
star it is possible to determine the angle at which the orbital plane is inclined (ASTROweb.) In this case a
more accurate radial velocity can be determined.
An important indicator that a compact body is a
neutron star rather than a black hole is the presence of x-ray busters. X-ray bursters occur when gas from
the companion star deposits gas onto the surface of the neutron star (Freedman and Kauffman p 571-572.) As
the newly arrived hydrogen is converted to helium it is deposited onto the surface of the neutron star. Once
the layer is about 1 metre thick the temperature can increase enough to cause helium fusion. The result is a
short lived thermonuclear flash that fades in seconds. As a black hole does not have a surface this can not
happen. Therefore, if x-ray bursters are present in a system a neutron star must be
present.
Some systems containing compact objects have
spectra signatures indicating that the compact object is a neutron star. As the material from the accretion
disk mixes with the atmosphere of the neutron star the resultant energy state changes should be reflected in
the black body radiation of the neutron star (ASTROweb.) This can be used to disprove a black body candidate
if the signature is present.
Case Study: Cygnus
X-1
The case of Cygnus X-1 is worth detailing as it
highlights may of the techniques used be astronomers to detect black holes. In 1971 the Uhuru x-ray satellite
detected an x-ray source unlike those known to astronomers at the time (Freedman and Kauffman p 586-587.)
This new source was highly variable with changes measure in the hundredths of a second. This interested
astronomers as any ‘flickering’ can not be quicker than the time for light to cross the object. The
implication was that the body was less than 3000km across. Figure 2 shows a model of the development of this
system.
Soon afterwards it was discovered that Cygnus X-1
periodically emitted bursts of radiation in radio frequencies. This radiation came from the same place in the
sky as the star HDE 226868. Spectroscopic investigations found that HDE 226868 was a B0 class star which do
not emit x-ray radiation.
Another part of the puzzle came from the periodic
Doppler shifting of the light from HDE 226868. The period of the shift was at 5.6 days. This type of periodic
shifting is indicative of binary system (LaSala, Charles, Smith et al, 1998) Due to the information gathered
about HDE 226868 it is thought that the star has of about 30 solar masses. Given this mass and the orbital
period (with use of Newton’s form of Kepler’s third law) the invisible companion of HDE 226868 is 7 solar
masses or more. The maximum limit of a neutron star is about 3 solar masses (Bemillard and McClintock 2006)
so the invisible partner must be a black hole. This system is likely to contain a 16 solar mass black hole
(ASTROweb.) The presence of radio emissions and a lack of negative indicators of a compact body surface
reinforces the conclusion that this system contains a black hole.
Conclusions
Binary star systems containing stellar-mass black
holes are varied and the distinction between a black hole and a neutron star within a system can be tricky to
determine. However, we have found that it is possible to gain enough information to be fairly sure that what
we see is the result of a stellar-mass black hole.
We detect black holes due to the dynamics of binary
systems containing these objects and the presence of an x-ray and radio source. We can use the absence of
certain indicators that the compact body has a surface. If a surface is present the object can not be a black
hole
Since most stars that we observe are contained in
binary systems it is likely that many more stellar black holes are still to be
located.
==========================================
References
ASTROweb: Astrophysics Spectator: Black Holes in X-ray Binary Systems, http://www.astrophysicsspectator.com/topics/generalrelativity/BlackHoleXRayBinary.html
(accessed 2 April 2009)
CAMBweb: Cambridge X-ray Astronomy:
Black Holes and X-ray binaries http://www-xray.ast.cam.ac.uk/xray_introduction/Blackholebinary.html
COSweb: SAO Cosmos: Black Holes http://astronomy.swin.edu.au/cms/astro/cosmos/B/Black+Hole
Freedman, R. A. & Kaufmann, W. J, III. 2007, Universe ,
8th ed.; New York: W.H. Freeman & Co.
HARVweb: Chandra
X-ray Observatory:Schematic of a Black Hole http://chandra.harvard.edu/resources/illustrations/blackholesXrays.html
LaSala, J, Charles. P.A. R.A.D.Smith,
Baucinska-Church, M and Church, M.J. 1998. Mon.Not.R.Astron.Soc, 301, 285,
Malzac, J. 2008. Mem.S.A.It, 79, 134
McClintock and Remillard, Black Hole Binaries, 2004. Preprint arXiv:
astro-ph/0306213v4
NASAweb: NASA:Chandra X-ray Astronomy http://www.nasa.gov/mission_pages/chandra/astronomy/index.html
NOVAweb: Nova Celestia: X-Ray binary star
systems http://www.novacelestia.com/space_art_binary/x-ray_binaries.html
Remillard, R A and McClintock J E. 2006. Preprint arXiv:
astro-ph/0606352v1.
|